I love Yellowstone National Park. I have visited countries as far east as Japan, followed the footsteps of Romans, looked up at the Leaning Tower of Pisa, experienced volcanoes from afar and close up, and touched glaciers. Yet I return to Yellowstone again and again, gazing at waterfalls and lakes but especially at the vivid rainbow colors of many of the park’s hot springs, geysers, mud pots and fumaroles.
These colors draw me in. They are produced by millions and millions of tightly packed bacterial cells enmeshed in a slimy matrix. Although individual bacteria are not visible to the naked eye, in this slime they form easily seen communities referred to as microbial mats, or “biofilms.” Through a microscope these films show remarkable three-dimensional structure, with microbes glued onto one another to form many levels of filaments, winding pathways and features resembling tiny towers. To me, they look like cities of slime, a pulsing metropolis with blocks and skyscrapers and streets that are busier than major avenues in Tokyo or New York. And you’ve seen biofilms, too: they form the thick and slimy buildup in your drains and the stubborn rings around your bathtub.
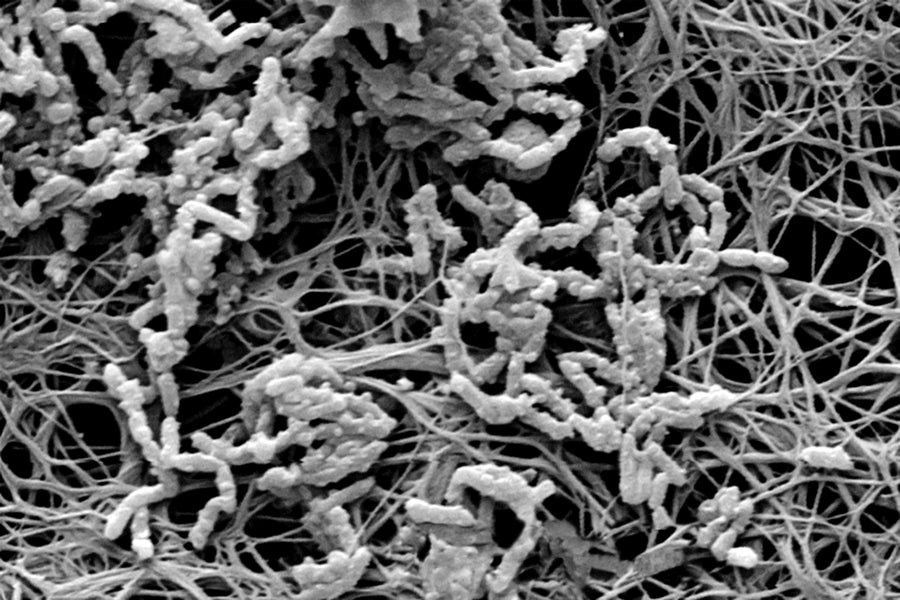
Micro menace: The insides of a medical catheter are covered with a bacterial biofilm (micrograph) that can cause blood infections. Credit: Getty Images
On supporting science journalism
If you're enjoying this article, consider supporting our award-winning journalism by subscribing. By purchasing a subscription you are helping to ensure the future of impactful stories about the discoveries and ideas shaping our world today.
Although this buildup is a nuisance, in medicine biofilms are a dire threat to our health. When bacteria succeed in forming a biofilm within people, they resist antibiotics and can be the culprits in chronic infections of surgical sites, lungs and urinary tracts. Biofilms can colonize medical devices and implants such as catheters, prosthetic joints and heart valves. Overall, 65 percent of hospital-acquired infections are caused by bacteria growing as biofilms. There are 1.7 million of these infections annually in U.S. hospitals alone and 99,000 associated deaths, so that is a tremendous amount of harm. Biofilms are thought to claim as many lives as cancer every year—a grim statistic indeed.
Why are bioflims such a problem in medicine? Conventional therapies, geared toward treating infections by single bacteria, have proved inadequate in the treatment of many (if not most) biofilm infections. This is because bacteria growing as biofilms not only have an extreme capacity for evading our host defenses and immune responses but also demonstrate an extraordinary tolerance to antibiotics. This resilience of biofilms is not linked to bacteria having acquired drug resistance or becoming the “superbugs” that we hear so much about these days. Instead it is the 3-D structure that makes biofilms so hardy and difficult to eliminate. Within it, bacteria communicate, cooperate and exchange material to not only organize their architectural features but manufacture proteins and other substances to support and protect one another.
There were early clues that bacteria within biofilms become fundamentally different from single cells. In 1998 researchers George A. O’Toole and Roberto Kolter demonstrated that biofilm formation by the soil bacterium Pseudomonas fluorescens required the synthesis of new proteins as well as the presence of 24 genes. Most of the genes were of unknown function, although some encoded proteins used for surface attachment, such as adhesins. The mystery genes suggested that becoming an attached cell meant taking on a novel bacterial physiology. Then, in 2002, my colleagues and I demonstrated that bacteria not only change on surface contact but keep transforming and adapting as the biofilm develops from just a few attached cells into a 3-D community, producing different sets of proteins at each stage. Further work showed that the proteins enable the transition from one biofilm stage to the next in a set sequence.
These findings suggested that biofilms, like cities, are built brick by brick, with their construction following a master plan, one building phase and one city block at a time. Knowing how biofilms are built means that we have started to understand how to interfere with the master plan. In the laboratory, by adding chemicals that inhibit or enhance some of these proteins, we can stop biofilms at a particular developmental stage or even remodel them, making them revert to earlier stages. And some strategies are making their way to the clinic.
An unhealthy attachment
Preventing bacterial attachment in the first place is a good place to start. Much of this research has focused on the development of surface materials or coatings capable of killing bacteria on contact. That can be accomplished using surface coatings or impregnated surfaces that enable local delivery of antibacterial agents at high concentrations. Several such surfaces are already commonplace in hospitals and include antibiotic-impregnated sutures and bone cement containing antibiotic-impregnated beads, as well as catheters, wound dressings, and endotracheal tubes coated with colloidal silver or silver nanoparticles. The silver ions kill bacteria on contact. The killing mechanism is not fully understood, but we do know that silver ions cause oxidative damage (where oxygen atoms pull electrons away from a microorganism’s essential biomolecules), leading to bacterial cell death. In addition to silver, metal oxide and metal salts—iron, mercury, tellurium, zinc, titanium—are being tested for clinical purposes.
Surface coatings and impregnated surfaces, however, have an Achilles’ heel because the antimicrobial substance in the coatings eventually run out. Combined with the concerns of overuse of drugs and compounds that are aimed at killing bacteria (including silver) and the consequent emergence of bacterial resistance, this has spawned the development of new surface materials that control attachment in more mechanical ways. The surfaces are inspired by nature, emulating the texture of shark skin, or self-cleaning textures found in lotus leaves, or the chemical functions used by mussels to repel bacteria. Bioinspired surfaces do not necessarily prevent bacteria from attaching but instead interfere with the proteins they use as attachment platforms. This process works by changing, at a microscopic level, surface roughness. That can be done by adding nanostructures such as brushes, crystals and tubules made of polymers that are attracted to water molecules, such as polyethylene glycol (PEG, inspired by mussels), and compounds known as zwitterionic polymers that were inspired by the antibiofouling properties of blood cells.
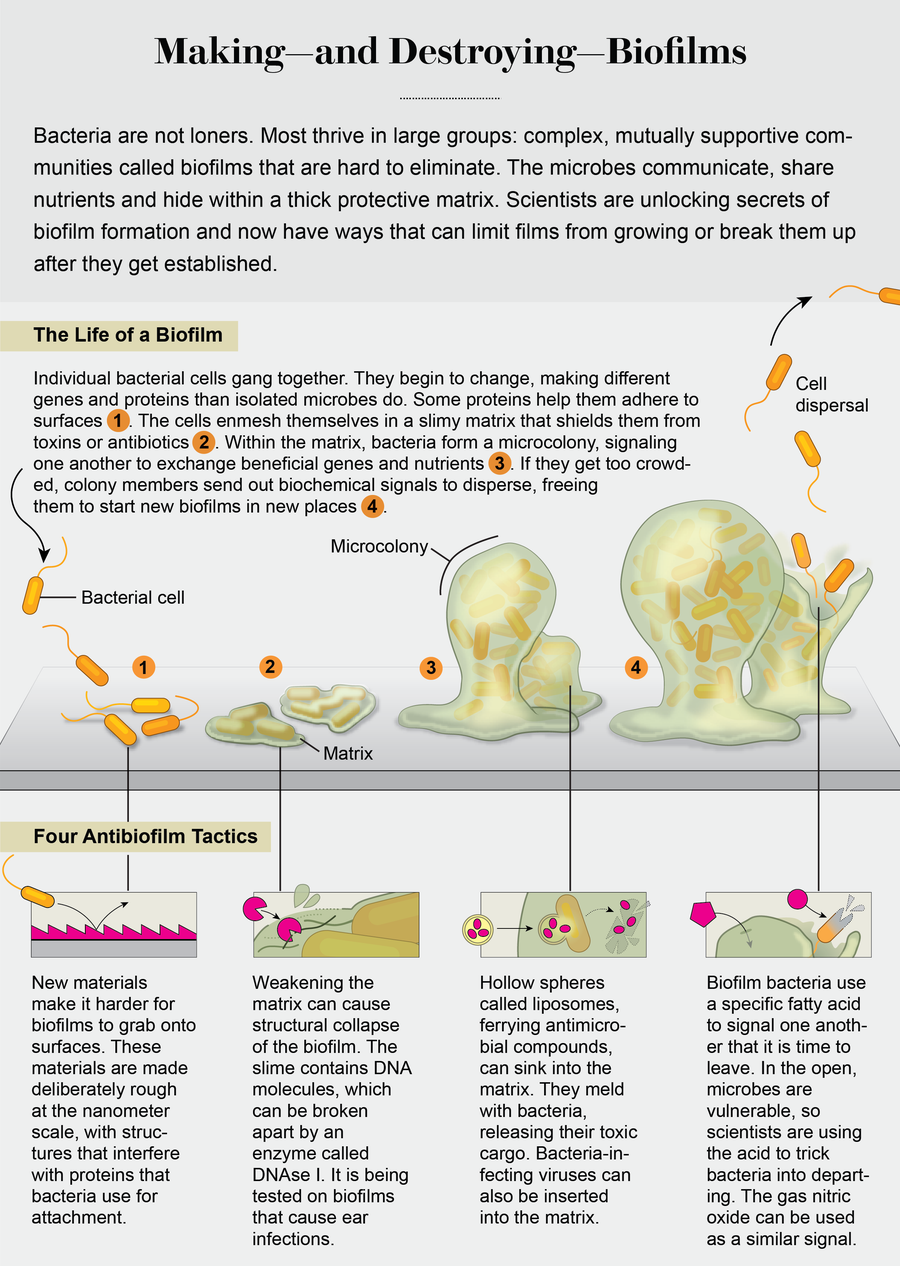
Credit: Jen Christiansen
The nanostructures can be arranged at varying distances to reduce bacterial attachment to different degrees. Some of these bioinspired surfaces have now been widely used in clinics. Others are still at the proof-of-concept stage, with hurdles such as manufacturing limitation and toxicity issues to be overcome.
Attack the matrix
Despite the promise of antibiofilm surfaces for medical implants, very different strategies are needed to treat biofilms that overcome such antiattachment strategies and form in dangerous places (in the absence of man-made surfaces), such as in wounds or lungs. In such cases, the slimy matrix surrounding biofilms becomes the target. This matrix, composed of long strands of sugar molecules called polysaccharides, proteins and DNA, helps the sheets of microbes in several ways. For one, the matrix functions as a protective layer. It blocks or restricts some antibiotics or immune system markers known as antibodies from reaching the bacteria within the biofilm structure. In addition, the matrix serves as a structural framework that glues the bacterial community together and to the surface. Thus, removal of this framework can result in loss of structural integrity and subsequent collapse, as the large bacterial gang breaks up into smaller cell clusters or individual cells. When that happens, the liberated bacterial cells become vulnerable once again to drugs and the immune system.
The downside of targeting the structural framework is that the composition of the biofilm matrix, more specifically the type of proteins and polysaccharides present in the matrix, varies greatly among bacteria, with each type requiring a specific degradative enzyme. Therefore, treatments aimed at the degradation of proteins and polysaccharides need to be tailored to the type of bacterium that forms the biofilm. Recent findings by Kendra Rumbaugh of Texas Tech University and colleagues suggests that this hurdle may be overcome using glycoside hydrolases, enzymes that target common, highly conserved glycosidic linkages present in polysaccharides. The team demonstrated that glycoside hydrolases effectively disrupt Staphylococcus aureus and Pseudomonas aeruginosa monoculture and coculture biofilms, leaving the disintegrated biofilm cells more susceptible to conventional antimicrobials. Follow-up tests in animals suggest that such therapies could potentially prove effective against a wide range of pathogens with diverse matrix compositions.
Another common matrix component may be DNA that appears to be universally present in biofilms. The matrix DNA is susceptible to degradation by one enzyme alone: DNAse I. Several clinical trials are focusing on the use of this substance; it is being evaluated for the treatment of chronic middle ear (otitis media) and other biofilm infections in combination with antibiotics. DNAse I is already used in the treatment of cystic fibrosis patients with early lung disease, with treatment coinciding with significantly improved lung function. (In this case, however, the enzyme seems to be reducing the stickiness of the sputum, thus enhancing lung clearance and antibiotic efficacy rather than inducing a collapse of the biofilms.)
Sound the retreat
A strategy of a different nature has been gleaned from watching the way in which biofilms develop. After bacteria form these films, they can disassemble them via a process called dispersion. Dispersion enables biofilms to revert to a single cell state and occurs when resources within them, such as nutrients, become exhausted, or when biofilms become too overcrowded, or when the outside environment becomes unstable. Breaking up can help biofilm members survive and spawn new communities at other locations.
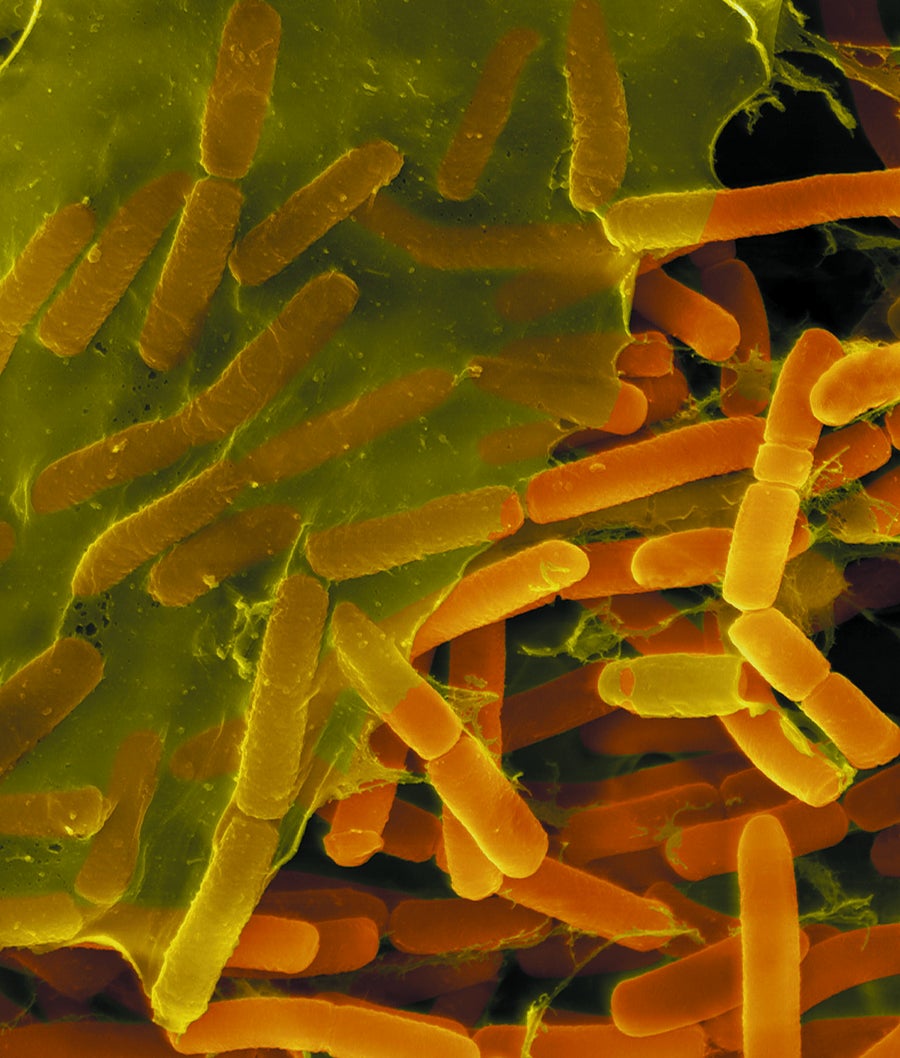
Togetherness: Bacillus bacteria (oblong shapes in micrograph) cover themselves in a protective matrix. Credit: Dennis Kinkel Science Source
Single cells leaving a biofilm are, however, exactly the kinds of unprotected bacteria we are good at treating with medicine. So how can we redirect film-forming bacteria to switch their lifestyle and escape from the biofilm? Researchers have identified several environmental growth conditions and compounds capable of inducing the switch, including exposure to heavy metals, availability of nutrients and oxygen, and presence of signaling molecules such as nitric oxide and a small fatty acid molecule named cis-2-decenoic acid (cis-DA). While these dispersion triggers are quite diverse, they all have in common that they induce dispersion by lowering the level of a universal intracellular signaling molecule named cyclic di-GMP. Levels of cyclic di-GMP determine the stickiness of bacteria to surfaces, with high levels being linked to surface-associated biofilm growth and matrix production and low levels being linked to bacteria growing as single cells. Although changes to cyclic di-GMP levels in response to these triggers have been linked with 80 percent or more of the biofilm biomass being removed, not all dispersion triggers are suitable for use in medical settings.
One viable candidate to emerge is the colorless gas nitric oxide. Our own immune system makes use of nitric oxide to fend off bacterial invaders, and it is already used medically to improve oxygenation in patients who have various forms of pulmonary hypertension (for example, chronic obstructive pulmonary disease). Lab studies have shown that nitric oxide mediates biofilm dispersal across a broad range of bacteria. Nitric oxide alone reduces biofilms on average by 63 percent. When combined with antimicrobial compounds such as colistin, the oxide was able to almost completely remove biofilms in lab experiments.
Still, despite the promising results, nitric oxide poses some clinical challenges. It can be toxic if it spreads to different areas of the body, so delivery and restriction to a specific site of infection are important—and hard to do with a gas. To better address some of the concerns, various formulations and devices have been developed. One example is cephalosporin-3′-diazeniumdiolates. This combination drug is composed of an antibiotic (cephalosporin) and a nitric oxide–producing substance that activates only on contact with bacterial cells that harbor an enzyme called β-lactamase. Bacteria resistant to antibiotics such as penicillin and ampicillin typically have this enzyme.
Research on P. aeruginosa, a model bacterium for biofilm formation and the cause of a large number of hospital-acquired and chronic infections, has shown that bacteria have their own way of telling members in the biofilm community to disperse. This bacterium produces cis-DA, which enables the organism to signal to its community members that it is time to leave. The ability of cis-DA to signal dispersion is not limited to P. aeruginosa biofilms. Instead cis-DA has been found to signal biofilms formed by other bacteria as well as yeast—not P. aeruginosa—to break up. There is also evidence that other bacteria produce a different version of this fatty acid, which means that biofilm bacteria may use particular “dialects” to tell members to disperse. Though still at the lab stage of testing, using such signaling and communication molecules in combination with antibiotics may very likely represent a future treatment strategy.
A recent study furthermore suggested the availability of pyruvate, a key intermediate in several metabolic pathways, to act as a toggle switch between the planktonic and biofilm lifestyle—high levels of pyruvate seemingly enhancing biofilm formation and depleted pyruvate inducing dispersion. In lab experiments, researchers used enzymes to deplete pyruvate, resulting in dispersion by the two pathogens P. aeruginosa and S. aureus. Moreover, when combined with antimicrobial compounds, such pyruvate-depleting conditions enhanced the potential of antibiotics such as tobramycin in killing biofilms. The combination strategy was also found to be effective in animal studies when tested on burn wounds infected with P. aeruginosa. In fact, the combination treatment was found to be more effective in eradicating biofilms present in burn wounds than the more commonly used antibacterial cream silver sulfadiazine.
Detect and infect
Another strategy to combat biofilms is to cause a lethal infection of their bacterial constituents. Like people, bacteria are susceptible to viruses, which in the case of these microbes are referred to as phages. Yet while people are not affected by phages, bacteria will be either permanently infected or killed. Since early studies in 1996 examining the interaction of phages with biofilms, research has aimed at identifying phages capable of killing bacteria within a biofilm. Moreover, phages that enter but do not kill bacterial cells can still be used as cargo trucks to deliver an antibiotic or a matrix-degrading agent (such as DNAse I) to each and every bacterium within the biofilm. Phage therapy is currently being used outside the U.S. in the treatment of biofilm-derived lung infections by cystic fibrosis patients.
But the therapy, like other tactics, comes with its own set of problems. Because phages infect and kill bacteria with high specificity, a particular phage infects only a specific type of bacterium, meaning they cannot be used as broad-spectrum killers. And bacteria can become resistant to phage attack, just like they develop resistance to antibiotics.
To overcome potential resistance, researchers have developed liposomes, or lipid-enclosed vesicles, as alternative cargo carriers. Referred to as nanoparticles because of their small size, the idea behind these mini transporters is to deliver compounds that kill biofilm cells or destabilize biofilms such as antibiotics, antibacterial compounds or matrix-degrading enzymes directly to where they are needed, into the biofilm or to the bacteria themselves. The targeting mechanism is based on lipids in the liposomes that mimic the lipids on bacterial cell membranes. The similarity allows them to diffuse into the matrix. Here lies the beauty of the system. The matching lipids enable liposomes to fuse with a bacterium and thus enable the transport system to directly spill its cargo into the microbe in a manner similar to an injection. An advantage of this strategy is the targeted approach because antibacterial and antibiofilm compounds are delivered only to the biofilm and nowhere else. Liposomes are already a very widely used antimicrobial drug-delivery system.
Winning combination
The tactics described here barely scratch the surface of the sheer number of strategies that are being tested in labs worldwide, some at early stages of development, some at the preclinical stage. But these efforts not only indicate the importance of biofilm control but also how challenging it is to truly get a handle on slime, mainly because all biofilms are not the same. Each bacterial species makes and escapes from biofilms in slightly different ways, using variations of communication molecules, producing different proteins and more. Yet as the approaches indicate, we are getting closer to a treatment that can help people, although such treatment may take advantage of a combination of antibiofilm strategies to demolish the bacterial cities that threaten our lives.